Nano-FTIR

Nano-FTIR (nanoscale Fourier transform infrared spectroscopy) is a scanning probe technique that utilizes as a combination of two techniques: Fourier transform infrared spectroscopy (FTIR) and scattering-type scanning near-field optical microscopy (s-SNOM). As s-SNOM, nano-FTIR is based on atomic-force microscopy (AFM), where a sharp tip is illuminated by an external light source and the tip-scattered light (typically back-scattered) is detected as a function of tip position. A typical nano-FTIR setup thus consists of an atomic force microscope, a broadband infrared light source used for tip illumination, and a Michelson interferometer acting as Fourier-transform spectrometer. In nano-FTIR, the sample stage is placed in one of the interferometer arms, which allows for recording both amplitude and phase of the detected light (unlike conventional FTIR that normally does not yield phase information). Scanning the tip allows for performing hyperspectral imaging (i.e. complete spectrum at every pixel of the scanned area) with nanoscale spatial resolution determined by the tip apex size. The use of broadband infrared sources enables the acquisition of continuous spectra, which is a distinctive feature of nano-FTIR compared to s-SNOM. Nano-FTIR is capable of performing infrared (IR) spectroscopy of materials in ultrasmall quantities and with nanoscale spatial resolution.[1] The detection of a single molecular complex[2] and the sensitivity to a single monolayer[3] has been shown. Recording infrared spectra as a function of position can be used for nanoscale mapping of the sample chemical composition,[4][5] performing a local ultrafast IR spectroscopy[6] and analyzing the nanoscale intermolecular coupling,[7] among others. A spatial resolution of 10 nm to 20 nm is routinely achieved.[4]

For organic compounds, polymers, biological and other soft matter, nano-FTIR spectra can be directly compared to the standard FTIR databases, which allows for a straightforward chemical identification and characterization.[4]
Nano-FTIR does not require special sample preparation and is typically performed under ambient conditions. It uses an AFM operated in noncontact mode that is intrinsically nondestructive and sufficiently gentle to be suitable for soft-matter and biological sample investigations. Nano-FTIR can be utilized from THz to visible spectral range (and not only in infrared as its name suggests) depending on the application requirements and availability of broadband sources. Nano-FTIR is complementary to tip-enhanced Raman spectroscopy (TERS), SNOM, AFM-IR and other scanning probe methods that are capable of performing vibrational analysis.
Basic principles
[edit]
Nano-FTIR is based on s-SNOM, where the infrared beam from a light source is focused onto a sharp, typically metalized AFM tip and the backscattering is detected. The tip greatly enhances the illuminating IR light in the nanoscopic volume around its apex, creating a strong near field. A sample, brought into this near field, interacts with the tip electromagnetically and modifies the tip (back)scattering in the process. Thus by detecting tip scattering, one can obtain information about the sample.
Nano-FTIR detects the tip-scattered light interferometrically. The sample stage is placed into one arm of a conventional Michelson interferometer, while a mirror on a piezo stage is placed into another, reference arm. Recording the backscattered signal while translating the reference mirror yields an interferogram. The subsequent Fourier transform of this interferogram returns the near-field spectra of the sample.
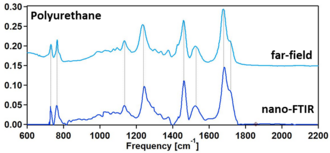
Placement of the sample stage into one of the interferometer's arms (instead of outside of the interferometer as typically implemented in conventional FTIR) is a key element of nano-FTIR. It boosts the weak near-field signal due to interference with the strong reference field, helps to eliminate the background caused by parasitic scattering off everything that falls into large diffraction-limited beam focus, and most importantly, allows for recording of both amplitude s and phase φ spectra of the tip-scattered radiation.[8] With the detection of phase, nano-FTIR provides complete information about near fields, which is essential for quantitative studies and many other applications. For example, for soft matter samples (organics, polymers, biomaterials, etc.), φ directly relates to the absorption in the sample material.[9][10] This permits a direct comparison of nano-FTIR spectra with conventional absorption spectra of the sample material,[4] thus allowing for simple spectroscopic identification according to standard FTIR databases.
History
[edit]Nano-FTIR was first described in 2005 in a patent by Ocelic and Hillenbrand as Fourier-transform spectroscopy of tip-scattered light with an asymmetric spectrometer (i.e. the tip/sample placed inside one of the interferometer arms).[11] The first realization of s-SNOM with FTIR was demonstrated in 2006 in the laboratory of F. Keilmann using a mid-infrared source based on a simple version of nonlinear difference-frequency generation (DFG).[12] However, the mid-IR spectra in this realization were recorded using dual comb spectroscopy principles,[13][14] yielding a discrete set of frequencies and thus demonstrating a multiheterodyne imaging technique rather than nano-FTIR. The first continuous spectra were recorded only in 2009 in the same laboratory using a supercontinuum IR beam also obtained by DFG in GaSe upon superimposing two pulsed trains emitted from Er-doped fiber laser.[1] This source further allowed in 2011 for the first assessment of nanoscale-resolved spectra of SiC with excellent quality and spectral resolution.[15] At the same time, Huth et al.[16] in the laboratory of R. Hillenbrand used IR radiation from a simple glowbar source in combination with the principles of Fourier-transform spectroscopy, to record IR spectra of p-doped Si and its oxides in a semiconductor device. In the same work the term nano-FTIR was first introduced. However, an insufficient spectral irradiance of glowbar sources limited the applicability of the technique to the detection of strongly-resonant excitations such phonons;[17] and the early supercontinuum IR laser sources, while providing more power, had very narrow bandwidth (<300 cm−1). Further attempt to improve the spectral power, while retaining the large bandwidth of a glowbar source was made by utilizing the IR radiation from a high temperature argon arc source (also known as plasma source).[18][19] However, due to lack of commercial availability and rapid development of the IR supercontinium laser sources, plasma sources are not widely utilized in nano-FTIR.

The breakthrough in nano-FTIR came upon the development of high-power broadband mid-IR laser sources, which provided large spectral irradiance in a sufficiently large bandwidth (mW-level power in ~1000 cm-1 bandwidth)[21][22] and enabled truly broadband nanoscale-resolved material spectroscopy capable of detecting even the weakest vibrational resonances.[4][3][2][23] Particularly, it has been shown that nano-FTIR is capable of measuring molecular fingerprints which match well with far-field FTIR spectra, owing to the asymmetry of the nano-FTIR spectrometer that provides phase and thus gives access to the molecular absorption.[4] Recently, the first nanoscale-resolved infrared hyperspectral imaging of a co-polymer blend was demonstrated, which allowed for the application of statistical techniques such as multivariate analysis – a widely used tool for heterogeneous sample analysis.[20]
Additional boost to the development of nano-FTIR came from the utilization of the synchrotron radiation that provide extreme bandwidth, yet at the expense of weaker IR spectral irradiance compared to broadband laser sources.[24][25][26][27]
Commercialization
[edit]
The nano-FTIR technology has been commercialized by neaspec – a Germany-based spin-off company of the Max Planck Institute of Biochemistry founded by Ocelic, Hillenbrand and Keilmann in 2007 and based on the original patent by Ocelic and Hillenbrand.[11] The detection module optimized for broadband illumination sources was first made available in 2010 as a part of the standard neaSNOM microscope system. At this time, broadband IR-lasers have not been yet commercially available, however experimental broadband IR-lasers prove that the technology works perfect and that it has a huge application potential across many disciplines. The first nano-FTIR was commercially available in 2012 (supplied with still experimental broadband IR-laser sources), becoming the first commercial system for broadband infrared nano-spectroscopy. In 2015 neaspec develops and introduces Ultrafast nano-FTIR, the commercial version of ultrafast nano-spectroscopy. Ultrafast nano-FTIR is a ready-to-use upgrade for nano-FTIR to enable pump-probe nano-spectroscopy at best-in-class spatial resolution. The same year the development of a cryo-neaSNOM – the first system of its kind to enable nanoscale near-field imaging & spectroscopy at cryogenic temperatures – was announced.
Advanced capabilities
[edit]Synchrotron beamlines integration
[edit]Nano-FTIR systems can be easily integrated into synchrotron radiation beamlines. The use of synchrotron radiation allows for acquisition of an entire mid-infrared spectrum at once. Synchrotrons radiation has already been utilized in synchrotron infrared microscopectroscopy - the technique most widely used in biosciences, providing information on chemistry on microscales of virtually all biological specimens, like bone, plants, and other biological tissues.[28] Nano-FTIR brings the spatial resolution to 10-20 nm scale (vs. ~2-5 μm in microspectroscopy), which has been utilized for broadband spatially-resolved spectroscopy of crystalline[24][25] and phase-change[29] materials, semiconductors,[27] minerals,[30] biominerals and proteins.[26]
Ultrafast spectroscopy
[edit]Nano-FTIR is highly suitable for performing local ultrafast pump-probe spectroscopy due to intereferometric detection and an intrinsic ability to vary the probe delay time. It has been applied for studies of ultrafast nanoscale plasmonic phenomena in Graphene,[31][32] for performing nanospectroscopy of InAs nanowires with subcycle resolution[33] and for probing the coherent vibrational dynamics of nanoscopic ensembles.[6]
Quantitative studies
[edit]The availability of both amplitude and phase of the scattered field and theoretically well understood signal formation in nano-FTIR allow for the recovery of both real and imaginary parts of the dielectric function, i.e. finding the index of refraction and the extinction coefficient of the sample.[34] While such recovery for arbitrarily-shaped samples or samples exhibiting collective excitations, such as phonons, requires a resource-demanding numerical optimization, for soft matter samples (polymers, biological matter and other organic materials) the recovery of the dielectric function could often be performed in real time using fast semi-analytical approaches. One of such approaches is based on the Taylor expansion of the scattered field with respect to a small parameter that isolates the dielectric properties of the sample and allows for a polynomial representation of measured near-field contrast. With an adequate tip-sample interaction model[35] and with known measurement parameters (e.g. tapping amplitude, demodulation order, reference material, etc.), the sample permittivity can be determined as a solution of a simple polynomial equation[36]
Subsurface analysis
[edit]Near-field methods, including nano-FTIR, are typically viewed as a technique for surface studies due to short probing ranges of about couple tip radii (~20-50 nm). However it has been demonstrated that within such probing ranges, s-SNOM is capable of detecting subsurface features to some extents,[37][38][39][40] which could be used for the investigations of samples capped by thin protective layers,[41] or buried polymers,[42][43] among others.
As a direct consequence of being quantitative technique (i.e. capable of highly reproducible detection of both near-field amplitude & phase and well understood near-field interaction models), nano-FTIR also provides means for the quantitative studies of the sample interior (within the probing range of the tip near field, of course). This is often achieved by a simple method of utilizing signals recorded at multiple demodulation orders naturally returned by nano-FTIR in the process of background suppression. It has been shown that higher harmonics probe smaller volumes below the tip, thus encoding the volumetric structure of a sample.[44] This way, nano-FTIR has a demonstrated capability for the recovery of thickness and permittivity of layered films and nanostructures,[44] which has been utilized for the nanoscale depth profiling of multiphase materials[45] and high-Tc cuprate nanoconstriction devices patterned by focused ion beams.[46] In other words, nano-FTIR has a unique capability of recovering the same information about thin-film samples that is typically returned by ellipsometry or impedance spectroscopy, yet with nanoscale spatial resolution. This capability proved crucial for disentangling different surface states in topological insulators.[47]
Operation in liquid
[edit]Nano-FTIR uses scattered IR light to obtain information about the sample and has the potential to investigate electrochemical interfaces in-situ/operando and biological (or other) samples in their natural environment, such as water. The feasibility of such investigations has already been demonstrated by acquisition of nano-FTIR spectra through a capping Graphene layer on top of a supported material or through Graphene suspended on a perforated silicon nitride membrane (using the same s-SNOM platform that nano-FTIR utilizes).[48][49]
Cryogenic environment
[edit]Reveling the fundamentals of phase transitions in superconductors, correlated oxides, Bose-Einstein condensates of surface polaritons, etc. require spectroscopic studies at the characteristically nanometer length scales and in cryogenic environment. Nano-FTIR is compatible with cryogenic s-SNOM that has already been utilized for reveling a nanotextured coexistence of metal and correlated Mott insulator phases in Vanadium oxide near the metal-insulator transition.[50]
Special atmosphere environments
[edit]Nano-FTIR can be operated in different atmospheric environments by enclosing the system into an isolated chamber or a glove box. Such operation has already been used for the investigation of highly reactive Lithium-ion battery components.[45]
Applications
[edit]Nano-FTIR has a plenitude of applications,[51] including polymers and polymer composites,[4] organic films,[52] semiconductors,[16][26][27][46] biological research (cell membranes, proteins structure, studies of single viruses),[2][26][53] chemistry and catalysis,[54] photochemistry,[55] minerals and biominerals,[53][26][30] geochemistry,[56] corrosion[57] and materials sciences,[5][23] low-dimensional materials,[58][32] photonics,[59][26] energy storage,[45] cosmetics, pharmacology and environmental sciences.[60]
Materials and chemical sciences
[edit]Nano-FTIR has been used for the nanoscale spectroscopic chemical identification of polymers[4] and nanocomposites,[20] for in situ investigation of structure and crystallinity of organic thin films,[52] for strain characterization and relaxation in crystalline materials[23] and for high-resolution spatial mapping of catalytic reactions,[54] among others.
Biological and pharmaceutical sciences
[edit]Nano-FTIR has been used for investigation of protein secondary structure, bacterial membrane,[26] detection and studies of single viruses and protein complexes.[26] It has been applied to the detection of biominerals in bone tissue.[53][26] Nano-FTIR, when coupled with THz light, can also be applied to cancer and burn imaging with high optical contrast.
Semiconductor industry and research
[edit]Nano-FTIR has been used for nanoscale free carrier profiling and quantification of free carrier concentration in semiconductor devices,[16] for evaluation of ion beam damage in nanoconstriction devices,[46] and general spectroscopic characterization of semiconductor materials.[27]
Theory
[edit]High-harmonic demodulation for background suppression
[edit]The nano-FTIR interferometrically detects light scattered from the tip-sample system, . The power at the detector can be written as[61]
where is the reference field. The scattered field can be written as
and is dominated by parasitic background scattering, , from the tip shaft, cantilever sample roughness and everything else which falls into the diffraction-limited beam focus. To extract the near-field signal, , originating from the "hot-spot" below the tip apex (which carries the nanoscale-resolved information about the sample properties) a small harmonic modulation of the tip height H (i.e. oscillating the tip) with frequency Ω is provided and the detector signal is demodulated at higher harmonics of this frequency nΩ with n=1,2,3,4,... The background is nearly insensitive to small variations of the tip height and is nearly eliminated for sufficiently high demodulation orders (typically ). Mathematically this can be shown by expanding and into a Fourier series, which yields the following (approximated) expression for the demodulated detector signal:
where is the complex-valued number that is obtained by combining the lock-in amplitude, , and phase, , signals, is the n-th Fourier coefficient of the near-field contribution and C. C. stands for the complex conjugate terms. is the zeroth-order Fourier coefficient of the background contribution and is often called the multiplicative background because it enters the detector signal as a product with . It cannot be removed by the high-harmonic demodulation alone. In nano-FTIR the multiplicative background is eliminated as described below.
Asymmetric FTIR spectrometer
[edit]To acquire a spectrum, the reference mirror is continuously translated while recording the demodulated detector signal as a function of the reference mirror position , yielding an interferogram . This way the phase of the reference field changes according to for each spectral component of the reference field and the detector signal can thus be written as[62]
where is the reference field at zero delay . To obtain the nano-FTIR spectrum, , the interferogram is Fourier-transformed with respect to . The second term in the above equation does not depend on the reference mirror position and after the Fourier transformation contributes only to the DC signal. Thus for only the near-field contribution multiplied by the reference field stays in the acquired spectrum:
This way, besides providing the interferometric gain, the asymmetric interferometer utilized in nano-FTIR also eliminates the multiplicative background, which otherwise could be a source of various artifacts and is often overlooked in other s-SNOM based spectroscopies.
Normalization
[edit]Following the standard FTIR practice, spectra in nano-FTIR are normalized to those obtained on a known, preferably spectrally-flat reference material. This eliminates the generally unknown reference field and any instrumental functions, yielding spectra of the near-field contrast:
Near-field contrast spectra are generally complex-valued, reflecting on the possible phase delay of the sample-scattered field with respect to the reference. Near-field contrast spectra depend nearly exclusively on the dielectric properties of sample material and can be used for its identification and characterization.
Nano-FTIR absorption spectroscopy
[edit]For the purpose of describing near-field contrasts for optically thin samples composed of polymers, organics, biological matter and other soft matter (so called weak oscillators), the near-field signal to a good approximation can be expressed as:[36]
,
where is the surface response function that depends on the complex-valued dielectric function of the sample and can be also viewed as the reflection coefficient for evanescent waves that constitute the near field of the tip. That is, the spectral dependence of is determined exclusively by the sample reflection coefficient. The latter is purely real and acquires an imaginary part only in narrow spectral regions around the sample absorption lines. This means that the spectrum of an imaginary part of the near-field contrast resembles the conventional FTIR absorbance spectrum, , of the sample material:[4]. It is therefore convenient to define the nano-FTIR absorption , which directly relates to the sample absorbance spectrum:
It can be used for direct sample identification and characterization according to the standard FTIR databases without the need of modeling the tip-sample interaction.
For phononic and plasmonic samples in the vicinity of the corresponding surface surface resonances, the relation might not hold. In such cases the simple relation between and can not be obtained, requiring modeling of the tip-sample interaction for spectroscopic identification of such samples.[40]
Analytical and numerical simulations
[edit]Significant efforts have been put towards simulating nano-FTIR electric field and complex scattering signal through numerical methods[63] (using commercial proprietary software such as CST Microwave Studio, Lumerical FDTD, and COMSOL Multiphysics) as well as through analytical models[64] (such as through finite dipole and point dipole approximations). Analytical simulations tend to be more simplified and inaccurate, while numerical methods are more rigorous but computationally expensive.
References
[edit]- ^ a b Amarie S, Ganz T, Keilmann F (November 2009). "Mid-infrared near-field spectroscopy". Optics Express. 17 (24): 21794–801. Bibcode:2009OExpr..1721794A. doi:10.1364/oe.17.021794. PMID 19997423.
- ^ a b c Amenabar I, Poly S, Nuansing W, Hubrich EH, Govyadinov AA, Huth F, et al. (2013-12-04). "Structural analysis and mapping of individual protein complexes by infrared nanospectroscopy". Nature Communications. 4: 2890. Bibcode:2013NatCo...4.2890A. doi:10.1038/ncomms3890. PMC 3863900. PMID 24301518.
- ^ a b Xu XG, Rang M, Craig IM, Raschke MB (July 2012). "Pushing the Sample-Size Limit of Infrared Vibrational Nanospectroscopy: From Monolayer toward Single Molecule Sensitivity". The Journal of Physical Chemistry Letters. 3 (13): 1836–41. doi:10.1021/jz300463d. PMID 26291869.
- ^ a b c d e f g h i j Huth F, Govyadinov A, Amarie S, Nuansing W, Keilmann F, Hillenbrand R (August 2012). "Nano-FTIR absorption spectroscopy of molecular fingerprints at 20 nm spatial resolution". Nano Letters. 12 (8): 3973–8. Bibcode:2012NanoL..12.3973H. doi:10.1021/nl301159v. PMID 22703339.
- ^ a b Ugarte L, Santamaria-Echart A, Mastel S, Autore M, Hillenbrand R, Corcuera MA, Eceiza A (2017-01-01). "An alternative approach for the incorporation of cellulose nanocrystals in flexible polyurethane foams based on renewably sourced polyols". Industrial Crops and Products. 95: 564–573. doi:10.1016/j.indcrop.2016.11.011. hdl:10810/64521.
- ^ a b Xu XG, Raschke MB (April 2013). "Near-field infrared vibrational dynamics and tip-enhanced decoherence". Nano Letters. 13 (4): 1588–95. Bibcode:2013NanoL..13.1588X. doi:10.1021/nl304804p. PMID 23387347.
- ^ Pollard B, Muller EA, Hinrichs K, Raschke MB (April 2014). "Vibrational nano-spectroscopic imaging correlating structure with intermolecular coupling and dynamics". Nature Communications. 5: 3587. Bibcode:2014NatCo...5.3587P. doi:10.1038/ncomms4587. PMC 4071972. PMID 24721995.
- ^ Huth F (2015). Nano-FTIR - Nanoscale Infrared Near-Field Spectroscopy (Ph.D.). Universidad del Pais Vasco.
- ^ Taubner T, Hillenbrand R, Keilmann F (2004-11-22). "Nanoscale polymer recognition by spectral signature in scattering infrared near-field microscopy". Applied Physics Letters. 85 (21): 5064–5066. Bibcode:2004ApPhL..85.5064T. doi:10.1063/1.1827334. ISSN 0003-6951.
- ^ Carney PS, Deutsch B, Govyadinov AA, Hillenbrand R (January 2012). "Phase in nanooptics". ACS Nano. 6 (1): 8–12. doi:10.1021/nn205008y. PMID 22214211.
- ^ a b WO patent 2007039210, Nenad Ocelic & Rainer Hillenbrand, "Optical device for measuring modulated signal light", published 2007-04-12
- ^ Brehm M, Schliesser A, Keilmann F (November 2006). "Spectroscopic near-field microscopy using frequency combs in the mid-infrared". Optics Express. 14 (23): 11222–33. Bibcode:2006OExpr..1411222B. doi:10.1364/OE.14.011222. PMID 19529536.
- ^ Keilmann F, Gohle C, Holzwarth R (July 2004). "Time-domain mid-infrared frequency-comb spectrometer". Optics Letters. 29 (13): 1542–4. Bibcode:2004OptL...29.1542K. doi:10.1364/OL.29.001542. PMID 15259740.
- ^ Coddington I, Newbury N, Swann W (2016-04-20). "Dual-comb spectroscopy". Optica. 3 (4): 414–426. Bibcode:2016Optic...3..414C. doi:10.1364/OPTICA.3.000414. ISSN 2334-2536. PMC 8201420. PMID 34131580.
- ^ Amarie S (2011-01-01). "Broadband-infrared assessment of phonon resonance in scattering-type near-field microscopy". Physical Review B. 83 (4): 045404. Bibcode:2011PhRvB..83d5404A. doi:10.1103/PhysRevB.83.045404.
- ^ a b c Huth F, Schnell M, Wittborn J, Ocelic N, Hillenbrand R (May 2011). "Infrared-spectroscopic nanoimaging with a thermal source". Nature Materials. 10 (5): 352–6. Bibcode:2011NatMa..10..352H. doi:10.1038/nmat3006. PMID 21499314.
- ^ Ishikawa M, Katsura M, Nakashima S, Ikemoto Y, Okamura H (May 2012). "Broadband near-field mid-infrared spectroscopy and application to phonon resonances in quartz". Optics Express. 20 (10): 11064–72. Bibcode:2012OExpr..2011064I. doi:10.1364/oe.20.011064. PMID 22565729.
- ^ Huth F, Chuvilin A, Schnell M, Amenabar I, Krutokhvostov R, Lopatin S, Hillenbrand R (March 2013). "Resonant antenna probes for tip-enhanced infrared near-field microscopy". Nano Letters. 13 (3): 1065–72. Bibcode:2013NanoL..13.1065H. doi:10.1021/nl304289g. PMID 23362918.
- ^ McIntosh AL, Wofford BA, Lucchese RR, Bevan JW (2001-12-01). "High resolution Fourier transform infrared spectroscopy using a high temperature argon arc source". Infrared Physics & Technology. 42 (6): 509–514. Bibcode:2001InPhT..42..509M. doi:10.1016/S1350-4495(01)00113-X.
- ^ a b c Amenabar I, Poly S, Goikoetxea M, Nuansing W, Lasch P, Hillenbrand R (February 2017). "Hyperspectral infrared nanoimaging of organic samples based on Fourier transform infrared nanospectroscopy". Nature Communications. 8: 14402. Bibcode:2017NatCo...814402A. doi:10.1038/ncomms14402. PMC 5316859. PMID 28198384.
- ^ Keilmann F, Amarie S (2012-04-17). "Mid-infrared Frequency Comb Spanning an Octave Based on an Er Fiber Laser and Difference-Frequency Generation". Journal of Infrared, Millimeter, and Terahertz Waves. 33 (5): 479–484. arXiv:1202.5845. Bibcode:2012JIMTW..33..479K. doi:10.1007/s10762-012-9894-x. ISSN 1866-6892. S2CID 25305889.
- ^ Hegenbarth R, Steinmann A, Mastel S, Amarie S, Huber AJ, Hillenbrand R, Sarkisov SY, Giessen H (2014). "High-power femtosecond mid-IR sources for s-SNOM applications". Journal of Optics. 16 (9): 094003. Bibcode:2014JOpt...16i4003H. doi:10.1088/2040-8978/16/9/094003. S2CID 49192831.
- ^ a b c Bensmann S, Gaußmann F, Lewin M, Wüppen J, Nyga S, Janzen C, et al. (September 2014). "Near-field imaging and spectroscopy of locally strained GaN using an IR broadband laser". Optics Express. 22 (19): 22369–81. Bibcode:2014OExpr..2222369B. doi:10.1364/oe.22.022369. PMID 25321708.
- ^ a b Hermann P, Hoehl A, Patoka P, Huth F, Rühl E, Ulm G (February 2013). "Near-field imaging and nano-Fourier-transform infrared spectroscopy using broadband synchrotron radiation". Optics Express. 21 (3): 2913–9. Bibcode:2013OExpr..21.2913H. doi:10.1364/oe.21.002913. PMID 23481749.
- ^ a b Peragut F, Brubach JB, Roy P, De Wilde Y (2014). "Infrared near-field imaging and spectroscopy based on thermal or synchrotron radiation". Applied Physics Letters. 104 (25): 251118. Bibcode:2014ApPhL.104y1118P. doi:10.1063/1.4885416. ISSN 0003-6951.
- ^ a b c d e f g h i Bechtel HA, Muller EA, Olmon RL, Martin MC, Raschke MB (May 2014). "Ultrabroadband infrared nanospectroscopic imaging". Proceedings of the National Academy of Sciences of the United States of America. 111 (20): 7191–6. Bibcode:2014PNAS..111.7191B. doi:10.1073/pnas.1400502111. PMC 4034206. PMID 24803431.
- ^ a b c d Hermann P, Hoehl A, Ulrich G, Fleischmann C, Hermelink A, Kästner B, et al. (July 2014). "Characterization of semiconductor materials using synchrotron radiation-based near-field infrared microscopy and nano-FTIR spectroscopy". Optics Express. 22 (15): 17948–58. Bibcode:2014OExpr..2217948H. doi:10.1364/oe.22.017948. PMID 25089414.
- ^ Marinkovic NS, Chance MR (2006-01-01). "Synchrotron infrared microspectroscopy". Reviews in Cell Biology and Molecular Medicine. Wiley-VCH Verlag GmbH & Co. KGaA. doi:10.1002/3527600906.mcb.200500021. ISBN 9783527600908.
- ^ Gilbert Corder SN, Chen X, Zhang S, Hu F, Zhang J, Luan Y, et al. (December 2017). "Near-field spectroscopic investigation of dual-band heavy fermion metamaterials". Nature Communications. 8 (1): 2262. Bibcode:2017NatCo...8.2262G. doi:10.1038/s41467-017-02378-3. PMC 5741627. PMID 29273808.
- ^ a b Hao Z, Bechtel HA, Kneafsey T, Gilbert B, Nico PS (February 2018). "Cross-Scale Molecular Analysis of Chemical Heterogeneity in Shale Rocks". Scientific Reports. 8 (1): 2552. Bibcode:2018NatSR...8.2552H. doi:10.1038/s41598-018-20365-6. PMC 5803189. PMID 29416052.
- ^ Wagner M, Fei Z, McLeod AS, Rodin AS, Bao W, Iwinski EG, et al. (February 2014). "Ultrafast and nanoscale plasmonic phenomena in exfoliated graphene revealed by infrared pump-probe nanoscopy". Nano Letters. 14 (2): 894–900. arXiv:1402.6003. Bibcode:2014NanoL..14..894W. doi:10.1021/nl4042577. PMID 24479682. S2CID 19561017.
- ^ a b Ni GX, Wang L, Goldflam MD, Wagner M, Fei Z, McLeod AS, et al. (2016). "Ultrafast optical switching of infrared plasmon polaritons in high-mobility graphene". Nature Photonics. 10 (4): 244–247. Bibcode:2016NaPho..10..244N. doi:10.1038/nphoton.2016.45. S2CID 4278267.
- ^ Eisele M, Cocker TL, Huber MA, Plankl M, Viti L, Ercolani D, et al. (2014). "Ultrafast multi-terahertz nano-spectroscopy with sub-cycle temporal resolution". Nature Photonics. 8 (11): 841–845. arXiv:1604.04304. Bibcode:2014NaPho...8..841E. doi:10.1038/nphoton.2014.225. S2CID 119285417.
- ^ Tranca DE, Stanciu SG, Hristu R, Stoichita C, Tofail SA, Stanciu GA (July 2015). "High-resolution quantitative determination of dielectric function by using scattering scanning near-field optical microscopy". Scientific Reports. 5: 11876. Bibcode:2015NatSR...511876T. doi:10.1038/srep11876. PMC 5155613. PMID 26138665.
- ^ Cvitkovic A, Ocelic N, Hillenbrand R (July 2007). "Analytical model for quantitative prediction of material contrasts in scattering-type near-field optical microscopy". Optics Express. 15 (14): 8550–65. Bibcode:2007OExpr..15.8550C. doi:10.1364/oe.15.008550. PMID 19547189.
- ^ a b Govyadinov AA, Amenabar I, Huth F, Carney PS, Hillenbrand R (May 2013). "Quantitative Measurement of Local Infrared Absorption and Dielectric Function with Tip-Enhanced Near-Field Microscopy". The Journal of Physical Chemistry Letters. 4 (9): 1526–31. CiteSeerX 10.1.1.666.8910. doi:10.1021/jz400453r. PMID 26282309.
- ^ Taubner T, Keilmann F, Hillenbrand R (October 2005). "Nanoscale-resolved subsurface imaging by scattering-type near-field optical microscopy". Optics Express. 13 (22): 8893–9. Bibcode:2005OExpr..13.8893T. doi:10.1364/opex.13.008893. PMID 19498922.
- ^ Jung L, Hauer B, Li P, Bornhöfft M, Mayer J, Taubner T (March 2016). "Exploring the detection limits of infrared near-field microscopy regarding small buried structures and pushing them by exploiting superlens-related effects" (PDF). Optics Express. 24 (5): 4431–4441. Bibcode:2016OExpr..24.4431J. doi:10.1364/oe.24.004431. PMID 29092272.
- ^ Krutokhvostov R, Govyadinov AA, Stiegler JM, Huth F, Chuvilin A, Carney PS, Hillenbrand R (January 2012). "Enhanced resolution in subsurface near-field optical microscopy". Optics Express. 20 (1): 593–600. Bibcode:2012OExpr..20..593K. doi:10.1364/oe.20.000593. PMID 22274381.
- ^ a b Zhang LM (2012-01-01). "Near-field spectroscopy of silicon dioxide thin films". Physical Review B. 85 (7): 075419. arXiv:1110.4927. Bibcode:2012PhRvB..85g5419Z. doi:10.1103/PhysRevB.85.075419. S2CID 37170378.
- ^ Lewin M, Hauer B, Bornhöfft M, Jung L, Benke J, Michel AK, et al. (2015-10-12). "Imaging of phase change materials below a capping layer using correlative infrared near-field microscopy and electron microscopy". Applied Physics Letters. 107 (15): 151902. Bibcode:2015ApPhL.107o1902L. doi:10.1063/1.4933102. ISSN 0003-6951.
- ^ Ho K, Kim KS, Gilburd L, Mirzoyan R, de Beer S, Walker GC (2019-05-10). "Nanoscale Subsurface Morphologies in Block Copolymer Thin Films Revealed by Combined Near-Field Infrared Microscopy and Mechanical Mapping". ACS Applied Polymer Materials. 1 (5): 933–938. doi:10.1021/acsapm.9b00189. S2CID 146790787.
- ^ Mester L, Govyadinov AA, Chen S, Goikoetxea M, Hillenbrand R (July 2020). "Subsurface chemical nanoidentification by nano-FTIR spectroscopy". Nature Communications. 11 (1): 3359. Bibcode:2020NatCo..11.3359M. doi:10.1038/s41467-020-17034-6. PMC 7335173. PMID 32620874.
- ^ a b Govyadinov AA, Mastel S, Golmar F, Chuvilin A, Carney PS, Hillenbrand R (July 2014). "Recovery of permittivity and depth from near-field data as a step toward infrared nanotomography". ACS Nano. 8 (7): 6911–21. doi:10.1021/nn5016314. hdl:11336/33709. PMID 24897380.
- ^ a b c Lucas IT, McLeod AS, Syzdek JS, Middlemiss DS, Grey CP, Basov DN, Kostecki R (January 2015). "IR near-field spectroscopy and imaging of single Li(x)FePO4 microcrystals". Nano Letters. 15 (1): 1–7. Bibcode:2015NanoL..15....1L. doi:10.1021/nl5010898. PMID 25375874.
- ^ a b c Gozar A, Litombe NE, Hoffman JE, Božović I (March 2017). "Optical Nanoscopy of High Tc Cuprate Nanoconstriction Devices Patterned by Helium Ion Beams". Nano Letters. 17 (3): 1582–1586. arXiv:1703.02101. Bibcode:2017NanoL..17.1582G. doi:10.1021/acs.nanolett.6b04729. PMID 28166407. S2CID 206737748.
- ^ Mooshammer F, Sandner F, Huber MA, Zizlsperger M, Weigand H, Plankl M, et al. (December 2018). "Nanoscale Near-Field Tomography of Surface States on (Bi0.5Sb0.5)2Te3" (PDF). Nano Letters. 18 (12): 7515–7523. Bibcode:2018NanoL..18.7515M. doi:10.1021/acs.nanolett.8b03008. PMID 30419748. S2CID 53292498.
- ^ Khatib O, Wood JD, McLeod AS, Goldflam MD, Wagner M, Damhorst GL, et al. (August 2015). "Graphene-Based Platform for Infrared Near-Field Nanospectroscopy of Water and Biological Materials in an Aqueous Environment". ACS Nano. 9 (8): 7968–75. arXiv:1509.01743. doi:10.1021/acsnano.5b01184. PMID 26223158. S2CID 30158736.
- ^ Lu YH, Larson JM, Baskin A, Zhao X, Ashby PD, Prendergast D, et al. (August 2019). "Infrared Nanospectroscopy at the Graphene-Electrolyte Interface". Nano Letters. 19 (8): 5388–5393. Bibcode:2019NanoL..19.5388L. doi:10.1021/acs.nanolett.9b01897. OSTI 1580901. PMID 31306028. S2CID 196812291.
- ^ McLeod AS, Van Heumen E, Ramirez JG, Wang S, Saerbeck T, Guenon S, et al. (2017). "Nanotextured phase coexistence in the correlated insulator V2O3". Nature Physics. 13 (1): 80–86. Bibcode:2017NatPh..13...80M. doi:10.1038/nphys3882.
- ^ Muller EA, Pollard B, Raschke MB (April 2015). "Infrared Chemical Nano-Imaging: Accessing Structure, Coupling, and Dynamics on Molecular Length Scales". The Journal of Physical Chemistry Letters. 6 (7): 1275–84. doi:10.1021/acs.jpclett.5b00108. PMID 26262987.
- ^ a b Westermeier C, Cernescu A, Amarie S, Liewald C, Keilmann F, Nickel B (June 2014). "Sub-micron phase coexistence in small-molecule organic thin films revealed by infrared nano-imaging". Nature Communications. 5: 4101. Bibcode:2014NatCo...5.4101W. doi:10.1038/ncomms5101. PMC 4082641. PMID 24916130.
- ^ a b c Amarie S, Zaslansky P, Kajihara Y, Griesshaber E, Schmahl WW, Keilmann F (2012-04-05). "Nano-FTIR chemical mapping of minerals in biological materials". Beilstein Journal of Nanotechnology. 3 (1): 312–23. doi:10.3762/bjnano.3.35. PMC 3343267. PMID 22563528.
- ^ a b Wu CY, Wolf WJ, Levartovsky Y, Bechtel HA, Martin MC, Toste FD, Gross E (January 2017). "High-spatial-resolution mapping of catalytic reactions on single particles". Nature. 541 (7638): 511–515. Bibcode:2017Natur.541..511W. doi:10.1038/nature20795. OSTI 1439214. PMID 28068671. S2CID 4452069.
- ^ Chen W, Qing G, Sun T (December 2016). "A novel aggregation-induced emission enhancement triggered by the assembly of a chiral gelator: from non-emissive nanofibers to emissive micro-loops". Chemical Communications. 53 (2): 447–450. doi:10.1039/c6cc08808b. PMID 27966702.
- ^ Dominguez G, Mcleod AS, Gainsforth Z, Kelly P, Bechtel HA, Keilmann F, et al. (December 2014). "Nanoscale infrared spectroscopy as a non-destructive probe of extraterrestrial samples". Nature Communications. 5: 5445. Bibcode:2014NatCo...5.5445D. doi:10.1038/ncomms6445. PMID 25487365.
- ^ Johnson CM, Böhmler M (2016-07-01). "Nano-FTIR microscopy and spectroscopy studies of atmospheric corrosion with a spatial resolution of 20 nm". Corrosion Science. 108: 60–65. doi:10.1016/j.corsci.2016.02.037.
- ^ Dai S, Fei Z, Ma Q, Rodin AS, Wagner M, McLeod AS, et al. (March 2014). "Tunable phonon polaritons in atomically thin van der Waals crystals of boron nitride". Science. 343 (6175): 1125–9. Bibcode:2014Sci...343.1125D. doi:10.1126/science.1246833. hdl:1721.1/90317. PMID 24604197. S2CID 4253950.
- ^ Li P, Lewin M, Kretinin AV, Caldwell JD, Novoselov KS, Taniguchi T, et al. (June 2015). "Hyperbolic phonon-polaritons in boron nitride for near-field optical imaging and focusing". Nature Communications. 6: 7507. arXiv:1502.04093. Bibcode:2015NatCo...6.7507L. doi:10.1038/ncomms8507. PMC 4491815. PMID 26112474.
- ^ Pletikapić G, Ivošević DeNardis N (2017-01-06). "Application of surface analytical methods for hazardous situation in the Adriatic Sea: monitoring of organic matter dynamics and oil pollution" (PDF). Nat. Hazards Earth Syst. Sci. 17 (1): 31–44. Bibcode:2017NHESS..17...31P. doi:10.5194/nhess-17-31-2017. ISSN 1684-9981.
- ^ Ocelic N, Huber A, Hillenbrand R (2006-09-04). "Pseudoheterodyne detection for background-free near-field spectroscopy". Applied Physics Letters. 89 (10): 101124. Bibcode:2006ApPhL..89j1124O. doi:10.1063/1.2348781. ISSN 0003-6951.
- ^ Huth F (2015). Nano-FTIR - Nanoscale Infrared Near-Field Spectroscopy (Ph.D.). Universidad del Pais Vasco.
- ^ Chen X, Lo CF, Zheng W, Hu H, Dai Q, Liu M (2017-11-27). "Rigorous numerical modeling of scattering-type scanning near-field optical microscopy and spectroscopy". Applied Physics Letters. 111 (22): 223110. Bibcode:2017ApPhL.111v3110C. doi:10.1063/1.5008663. ISSN 0003-6951.
- ^ Cvitkovic A, Ocelic N, Hillenbrand R (July 2007). "Analytical model for quantitative prediction of material contrasts in scattering-type near-field optical microscopy". Optics Express. 15 (14): 8550–65. Bibcode:2007OExpr..15.8550C. doi:10.1364/oe.15.008550. PMID 19547189.